Cite report
IEA (2020), Power Systems in Transition, IEA, Paris https://www.iea.org/reports/power-systems-in-transition, Licence: CC BY 4.0
Report options
Electricity security matters more than ever
Introduction
Electricity is essential for the prosperity of our societies
It would be very hard to imagine our modern societies without a secure supply of electricity. While it only accounts for a fifth of primary energy use today, it is indispensable for the 24/7 and increasingly digital economy. Recent difficulties caused by the Covid-19 pandemic remind us of the critical importance of electricity in all aspects of our lives, such as keeping medical equipment working in hospitals and IT systems available for teleworking and video conferencing. The impacts of an extended outage go far beyond the power system or the value of the lost energy purchase itself.
Electricity’s share of final energy consumption is set to grow. Having increased from 15% in 2000 to 20% today, it is set to grow to 24% by 2040 if countries stay on their present course as in the Stated Policies Scenario of the IEA World Energy Outlook. Efficient electrification of a range of energy uses could make electricity our most significant energy source. If countries turn towards a diverse, cost-effective mix in line with the Paris Agreement, as in the IEA Sustainable Development Scenario, the role of electricity becomes even stronger, reaching 31% of final energy consumption by 2040. While the share of electricity in final consumption is less than half that of oil today, it overtakes oil by 2040 in the Sustainable Development Scenario.
The growing share of electricity in final energy demand itself does not fully capture its importance. Electricity has critical linkages with other parts of the energy sector, particularly the oil and gas industry, and underpins the basic activities of the residential, commercial and industrial sectors. As electricity drives increased shares of heating, cooling, transport and many digital sectors of communication, finance, healthcare and others, so the need for adequate electricity security measures escalates.
The electricity system has to cope with a wide range of threats, old and new
Electricity security is often referred to using the term “security of supply” or the more literal phrase of “keeping the lights on”. The ultimate goal is to provide electricity to consumers reliably and at reasonable cost. Many threats exist to meeting this objective, ranging from equipment failure and fuel supply shortages, to operational planning failure, human error and deliberate attack. The IEA applies the following definition:
Electricity security is the electricity system’s capability to ensure uninterrupted availability of electricity by withstanding and recovering from disturbances and contingencies.
Electricity security brings together all actions taken – technical, economic and political – to maximise the degree of security in the context of the energy transition, cyber events and climate impacts, both short and long term.
Key electricity security terms and definitions
Term |
Definition |
---|---|
Adequacy |
The ability of the electricity system to supply the aggregate electrical demand within an area at all times under normal operating conditions. The precise definition of what qualifies as normal conditions and understanding how the system copes with other situations is key in policy decisions. |
Operational security |
The ability of the electricity system to retain a normal state or to return to a normal state after any type of event as soon as possible. |
Resilience |
The ability of the system and its component parts to absorb, accommodate and recover from both short-term shocks and long-term changes. These shocks can go beyond conditions covered in standard adequacy assessments. |
Sources: Based on European Commission JRC and IEA Electricity Security.
The themes covered in this report are rarely addressed through the same lens. From a detailed technical perspective, issues such as market design, system stability, cybersecurity or physical resilience may be addressed as separate disciplines. For policy makers they cover similar questions, including how reliability is defined as a measurable objective, which organisations carry which responsibility, and how appropriate incentives are given to the sector to ensure adequacy with a diverse generation mix and adequate transmission and distribution networks.
These themes also become ever more relevant because of related underlying trends. Electricity is expected to take an increasing share of total final energy consumption in the coming decades as we couple electricity with heating, transport and other sectors as part of our drive to decarbonisation. Especially in emerging economies, an enormous rise in demand is foreseen due to population and economic growth. All this puts electricity security higher than ever on the energy policy agenda.
Types of interruption to electricity supply
As electricity is a regulated good and most often designated as critical infrastructure, governments are generally held accountable for the reliability of power supply. Although the electricity system has always been designed and regulated with reliability and cost-effectiveness in mind, the first step to properly addressing risk is to understand the type, size and depth of different power interruptions. While all of them can have potentially deep economic and safety consequences, proper risk management requires a clear view of the stakes. For this purpose, it is essential to understand the type, causes and magnitude of damage caused by different types of power sector events:
Cascading blackouts/black system events occur when an initial outage causes the system to collapse from an increasing series of line overloads. These events affect all customers on the network, except those with back-up generation, during a period from hours to days before full restoration. Social damage is significant as a black system event affects many essential services, such as payment systems, telecommunications and traffic lights. These events are mostly due to equipment failure and simultaneous contingencies, and are very rarely related to lack of installed generation capacity. The Hokkaido blackout in 2018, due to an earthquake, and the South Australian blackout in 2016, a mix of severe storms and flawed interconnection standards, are recent examples.
The potential indirect impacts of blackouts are also enormous and include: transport disruption (the unavailability of trains and charging stations for electric vehicles), food safety issues (risk to the cold chain), problems related to public order (crime and riots), and loss of economic activity. This can lead to health and safety problems as well as substantial financial losses. In extreme cases where power outages relate to extreme natural events, loss of electricity supply exacerbates other recovery challenges, making restoration of the power system one of the earliest priorities.
Load shedding is the deliberate disconnection of electrical power in one part or parts of a power system. It is a preventive measure taken by system operators to maintain system balance when supply is currently or expected to be short of the amount needed to serve load plus reserves, after exhausting other options like calling on demand response, emergency supplies and imports. These are short-duration events lasting from minutes to a few hours where small amounts of energy are rationed to segments of consumers (1% to 2% of the unserved energy during a cascading blackout), while allowing loads that provide essential services to continue to be served. They are, from a consumer point of view, indistinguishable from other interruptions on the distribution grid. The anticipated, controlled and limited use of this extreme measure should be seen as an instrument to maintain security of supply.
Most current reliability standards target a level of supply that would expect a small amount of acceptable load shedding as a way to balance security of supply and economic considerations. For example, the Alberta system operator in Canada has needed to apply this type of interruption in three events between 2006 and 2013, with a total duration of 5.9 hours and an amount of energy well below the regulatory (reliability) standard. Even if load shedding results only in small amounts of energy not being served, it completely cuts power supply to certain groups of customers, creating an array of inconveniences. To share the damage and minimise the inconvenience, the power cuts are often “rolled”, switching from one customer block to the next. In the near future, digitalisation should allow power systems to phase out this small but drastic and inefficient way of rationing energy. This would cut energy only to non-essential appliances or storage-enabled devices, and maintain it for other uses where interruption would create more inconvenience, such as lifts.
Long rationing periods of electricity occur when system operators and governments have to limit power supplies on a planned basis because of large deficits of electricity supply to meet demand. This is possibly the most harmful type of power sector event that a society can face. Some long-duration rationing events have meant rationing as much of 4% to 10% of annual electricity consumption, creating large social and macroeconomic impacts. They include the one in Brazil in 2001 due to drought and an unsuitable investment framework. The large supply shock in Japan following the Great East Japan Earthquake also belongs to this category, when the government responded with a nationwide electricity conservation campaign that forced industry to make massive electricity demand shifts.
Many emerging economies such as Iraq and South Africa see their economic and social welfare severely impacted by recurrent periods of electricity rationing that can last many months. Although developed economies have solved this type of event due to sound investment frameworks, it remains a challenge for many developing economies. Nonetheless, developed economies should not take for this dimension for granted; investment frameworks need to be updated and resilient to new trends.
Recent examples of major disruption to power supplies
Type of event, location, year |
Key factors |
Extent of blackout |
---|---|---|
Load shedding, United Kingdom 2019 |
Lightning strike, operational security |
1 million people for around 40 minutes, aggravated by transport delays |
Load shedding, California 2020 |
Heatwave |
Rolling blackouts affecting up to 3 million people for up to 2.5 hours across several days |
Load shedding, California 2019 |
Wildfires |
Transmission lines shut down affecting 11% of PG&E customers for 3 days |
Blackouts, Ukraine 2015–16 |
Cyberattacks |
1-6 hours, 200 000+ people |
Black system event, Hokkaido 2018 |
Earthquake |
5.3 million people for 12-48 hours |
Long energy rationing period, Brazil 2001 |
Drought, inadequate investment framework |
20% of total energy consumption for 6 months |
New trends demand an update to electricity security frameworks
The power sector landscape is changing dramatically
After more than a century of rising electrification, most middle- and high-income countries have managed to reach high levels of electricity reliability. This achievement is the result of complex institutional frameworks often involving multiple institutions and stakeholders. These frameworks govern crucial aspects, from the planning of the physical infrastructure, setting the market and investment frameworks, to the secure operation of the system and preparedness for natural catastrophes.
Electricity security frameworks are the result of more than a century of experience, with a relatively stable set of technological choices and well-understood risks. But past experience, as characterised below, is not always enough to prepare for the future.
- Electricity was mostly provided by vertically integrated utilities with regional monopoly using dispatchable thermal and hydro power plants and centrally controlled transmission and distribution networks.
- Large rotating mass for power generation also provided system inertia. Power generators were controlled manually and not connected to digital networks.
- Regulation made a single entity responsible for the stable supply of electricity in the region and set the electricity tariff at a level sufficient to cover the normal rate of return from the invested asset. Under this system, utilities were able to invest in generation and network facilities with a high level of confidence about their return.
In an increasing number of countries and regions, these assumptions no longer apply.
Electricity market systems with regulated regional monopolies have been replaced by unbundled competitive systems.
Variable renewable sources like wind and solar PV have become cheaper than thermal power generation and are increasing their share of supply. This development is welcome as countries seek to decarbonise the electricity sector. Solar PV is one of the rare technology areas that is on track to achieve its sustainability goals. Wind and solar are indigenous energy sources, and their growth can reduce fossil fuel import bills for many countries.
At the same time, these new variable sources require flexibility in the system to cover their variability and more elaborate forecasting of their outputs. They are non-synchronous generators and do not bring in system inertia.
Variable renewables, solar PV in particular, are more distributed than conventional generators. Systems with distributed resources can be more resilient than centralised systems, but require operators to have greater situation awareness. Some resources are even behind the meter at consumers’ properties. Network operators increasingly rely on demand-side response as a key source of flexibility.
To manage such complex systems, the role of digital information technologies is increasing exponentially, exposing the electricity system to cyberthreats.
Governments and industry are promoting decarbonisation of energy systems to achieve sustainability goals such as climate change mitigation, but ongoing climate change is already leading to extreme weather events and is challenging the robustness and resilience of electricity supply infrastructure.
Businesses across the entire electricity supply chain need to invest in secure stable electricity supply in response to these drastic changes, but are challenged by increased uncertainty and complexity surrounding electricity systems.
For this reason, our report focuses on three aspects that will increasingly attract the attention of policy makers:
- a changing electricity mix driving new measures to ensure operational security and longer-term system adequacy
- emerging risks to cybersecurity
- the need for greater resilience against adverse impacts of climate change, including extreme weather events
This report provides policy makers with a structural review of the types of threats the system will be facing in the coming decades and how they can be managed with proper institutional measures, market design and technology.
The new power sector landscape will be shaped by a combination of factors: a growing role for variable renewables, stagnation or reduced contributions from traditional low-carbon sources such as nuclear and hydro, decreasing thermal fleets, further digitalisation of the economy, climate change, and others. The combination of these factors will alter the potential impact and likelihood of electricity supply interruptions. They may well put more pressure on certain areas of the electricity security framework, such as rules designed to bring investment into the sector, while changing the nature of traditional energy security concerns, such as fuel security. How the threat map changes will depend on the specific power mix, the policies in place and the external threats to each power system.
Electricity system trends and their potential impacts on various aspects of electricity security
Trend | Flexibility | Fuel security | Adequacy | Climate resilience | Cyber resilience | Simultaneous contingencies |
---|---|---|---|---|---|---|
Higher shares of variable renewable | ||||||
Smaller-fossil fired fleets | ||||||
Declining shares of low-carbon dispatchable (nuclear/hydro) | ||||||
Decentralisation (e.g. distributed generation, battery storage) | ||||||
Digitalisation (e.g. connectivity, automation) |
Impact on security: | Decreased: | Neutral: | Uncertain or depends on implementation: | Increased: | ||||
Relative importance | High: | Medium: | Low: |
Notes: Circle colour indicates the potential impacts on various security aspects, e.g. flexibility. Circle size indicates the relative level of importance for a specific security aspect. The intent of the table is to illustrate the potential impacts in a generalised way. Actual impacts in specific countries and states/provinces will depend on their context and circumstances, e.g. generation mix, existing infrastructure, geography, climate. Simultaneous contingencies are outages of generation and transmission assets which are unexpectedly affected at the same time by the same event, such
Secure supply of electricity requires many risk dimensions to be properly managed. From fuel availability and sufficient resources to cover peak demand and periods of stress, such as an unexpected plant outage, to the resources needed to ensure stable behaviour of the power system in real time, all these dimensions need to be considered and assessed. The table indicates how these dimensions can be affected by electricity system trends. Each dimension will be affected, sometimes in a positive manner, reducing the risks and increasing the set of tools available to maintain secure operation, but also potentially in a negative way. For example, in a country where the electricity mix is dominated by hydropower, growing reliance on solar PV could increase climate resilience and act as a good hedge against changing hydrology, but it may also require existing assets to be operated in a new, more flexible way.
A sound electricity security framework needs to map how these trends will alleviate certain security concerns while increasing others.
Ensuring security of supply requires proper governance
Most large interruptions have multiple causes, and therefore policy makers need to account for many different dimensions in the institutional framework that governs the power sector. This emphasises the need for rigorous analysis to underpin the decision-making behind ensuring reliability and more general security of supply.
As the electricity system continues to evolve due to the energy transition and emerging trends such as cyberthreats and climate change, governments and regulators will need to continue to update the legal and regulatory requirements on all stakeholders to ensure that electricity security is maintained in the face of these changes.
There are already cases of legislative changes to redefine frameworks, roles and responsibilities for various institutional actors in the electricity system to adapt to ongoing transformations in the power sector. The passage of the so-called EU Clean Energy Package is an attempt to restructure the institutional framework for EU electricity markets, including electricity security, in the face of ongoing changes to the fuel mix and increasing interconnectivity across electricity systems. It is a gradual evolution from earlier European legislative initiatives, or packages, for the electricity system published in 1996, 2003 and 2009. These started from an unbundling and market perspective, but increasingly cover security-related provisions.
Regulators, in particular, will have an important role to play in ensuring electricity security amid the energy transition. Either with changes to law or stemming from existing statutory authority, they will need to adjust market designs and standards to reflect greater variability of supply and demand. They will also need to account for new threats such as cybersecurity and climate change.
For example, to support the secure integration of renewables into the grid and manage risks stemming from the retirement of baseload generation and lower utilisation of other plants, the US Federal Energy Regulatory Commission issued Order 842 in February 2018. It requires all new generators (regardless of size or technology) to be capable of providing primary frequency response – a specific ancillary service used to cope with sudden changes in supply and demand – as a precondition for grid interconnection. Similarly, the European Union established a legally binding grid code in 2016 across all its member states that requires all new connections to have essential ancillary service capabilities. This paves the way for future operational rules and balancing markets with ever higher shares of VRE and distributed resources.
Integrated planning beyond jurisdictional borders is needed, involving a complex set of players
New and emerging threats to the reliability of power systems present challenges to electricity planning frameworks. This is true across market structures, ranging from competitive markets with extensive private-sector participation to more vertically integrated utility models. The implications of such developments for the electricity sector should be taken into consideration together with the fundamentals of generation, transmission and distribution.
In many jurisdictions, increasingly integrated and co‑ordinated planning frameworks have played a vital role in the cost-effective and secure transition to a new electricity mix. They increase transparency and provide information to market participants and to all stakeholders in general, informing project developers, grid operators and authorities. These frameworks are increasingly co-ordinating investment in generation and grids. They are also useful for foreseeing the potential outcome of low-probability but high-impact events. New approaches are emerging for co‑ordinated and integrated planning practices that expand the traditional scope:
- inter-regional planning across different jurisdictions and balancing areas
- integrated planning across a diversity of supply and demand resources (and other non-wire alternatives)
- integrated planning between the electricity sector and other sectors.
These new approaches are expanding and reaching into distribution networks, which have historically depended on power supplied by the transmission network. The situation is changing as more generation resources are added locally to the distribution network at low- and medium-voltage level. Where deployment of many smaller distributed plants is concentrated geographically, reverse flows from the distribution network up to the transmission level become increasingly common and must be managed securely. Most distribution networks are physically capable of managing two-way flows of power, although a number of upgrades and operational changes in voltage management and protection schemes can be necessary.
Closer co‑ordination between transmission system operators and distribution system operators is important for dealing with this change. Policy makers can help ensure that transmission and distribution planning processes are better integrated with generation planning, particularly as the latter begins to take system flexibility into consideration. Appropriate planning rules will play a crucial role in the expansion of the electricity sector and to foresee the impacts of low-probability but high-impact events, covering the grid, new generation, storage and other flexibility options.
Electricity security during the energy transition
The clean energy transition will bring a major structural change in the generation profile of electricity systems around the world. Variable renewable generation has already surged over the past decade, driven by cost reductions and favourable policy environments. The trend is set to continue and even accelerate in line with climate change objectives. At the same time, conventional power plants, notably those using coal, nuclear and hydro, are stagnating or declining. On the demand side, electrification will increase demand for electricity, even in a context of growing energy efficiency, requiring far greater levels of investment in power systems than we are witnessing today.
Moreover, technology and digitalisation are enabling a more active role for consumers in power systems, which are poised to become more decentralised in the coming years. The challenge this presents to policy makers and system planners is to ensure system security by putting in place appropriate policies, regulation and market design features to support resource adequacy. This includes the advancement of a diversity of low-carbon generation technologies and new flexibility resources such as demand response, storage, digitalisation and greater market interconnection to help meet the new challenges.
Low-carbon sources on the rise
The energy transition will bring sizeable growth in variable renewables
The energy transition being seen in many systems in the world will bring major changes in the way the system is operated. The most significant is the large-scale deployment of low-cost variable renewables.
Share of variable renewables in the global electricity mix, 2015-2024
OpenAlong with this large deployment of VRE, conventional power plants that provided the dominant proportion of power system flexibility in past decades are now retiring. The remaining conventional plants are mostly powered by natural gas, particularly in Europe and the United States. In Europe this is developing in parallel with decreasing domestic production of natural gas, leading to a closer link between natural gas supply security and electricity security.
To achieve emission reduction objectives, deployment of wind and solar PV will have to accelerate substantially and will become dominant sources in various parts of any interconnected system. Nonetheless, the IEA Sustainable Development Scenario points to a diverse supply mix where solar PV and wind are dominant in capacity, but are supported by other low-carbon generation technologies, demand response and storage, as well as digitalisation and market interconnection. Natural gas and coal will still play a role as well, particularly in developing economies like India. Not achieving the scenario’s levels of generation from other low-carbon technologies, such as nuclear and biomass, would result in additional costs and challenges. It would be extremely challenging for wind and solar technologies to further accelerate growth to such levels that they can compensate for a lack of other low-carbon generation. Moreover, the scenarios provide a purely techno-economic perspective, without considering social acceptance or political factors related to additional wind turbines, nuclear facilities, fossil fuel plants and new overhead transmission lines, all of which can further complicate achieving an optimal, low-carbon generation mix.
Low-carbon dispatchable sources, such as nuclear, see their role reduced in the energy landscape
Nuclear power plants are in decline at a global level despite being low-carbon, dispatchable, and, to some extent, flexible generation sources. The IEA Nuclear Power in a Clean Energy System analysis projected a Nuclear Fade Case, which explores what could happen over the coming decades in the absence of any additional investment in lifetime extensions or new projects. This case is increasingly aligning with what may very well happen in the coming decades in advanced economies. In the scenario, nuclear capacity in advanced economies would decline by two-thirds by 2040, from about 280 GW in 2018 down to just over 90 GW in 2040. The European Union would see the largest decline, with the share of nuclear in generation falling from 25% in 2018 to below 5% in 2040.
Global nuclear capacity in the New Policies Scenario vs a Nuclear Fade Case, 2015-2040
OpenOther sources such as biomass and biogas are expected to grow, but the pace of growth (from 2.0% of capacity to 2.2% globally in the period 2018-30 in the Stated Policies Scenario) would be much slower than the declines in coal and nuclear. New technologies, such as power plants equipped with carbon capture, use and storage, are progressing far less quickly than required to achieve a sustainable path. As a result, there is a risk that growth in other low-carbon technologies aside from wind and solar PV will be insufficient to compensate for coal and nuclear closures. Clean hydrogen is gaining momentum and has strong potential as a long-term energy storage source if it can be scaled up in the coming decade, as being promoted in various policy initiatives already.
The energy transition will change the diversity of every power system – changing their vulnerability to shocks
Until now, wind and solar PV have contributed positively to diversity in the generation mix. They are indigenous resources, which have therefore helped fuel-importing countries reduce their import bills and increase their self-sufficiency. A well-diversified generation mix, with contributions from wind and solar PV, can improve electricity security by mitigating risks arising from physical supply disruptions and fuel price fluctuations. Small-scale generators, such as distributed wind and solar PV, also have the potential to facilitate recovery from large-scale blackouts during the restoration process, while large thermal power plants take longer to resume normal operations since they need a large part of the system to be restored. These are clear examples of electricity security benefits from increasing the share of wind and solar PV. For example, in the People’s Republic of China, a shift from a strong reliance on coal to increased wind and solar will increase the diversity of the generation mix out to 2040.
Looking ahead, electricity supply systems in some regions could see less diversity in power generation sources. European electricity markets have achieved a high level of interconnection between power systems across many countries. They are endowed with diverse power generation sources, including natural gas, coal, nuclear, hydro, wind, solar PV and other sources. Such generation fuel diversity has been a source of confidence in electricity supply security spanning a wide region. As some generation sources are likely to decline in capacity, the diversity of the future system will need to be found in a low-carbon generation mix, flexibility in supply and demand response, and the ever-increasing importance of grid interconnections.
Coal-fired power plants, in particular, are being decommissioned to align with ambitions to reduce CO2 emissions and pollution levels. The trend will need to continue in order to achieve climate change mitigation objectives, increasingly supported by policy measures and finance strategies to phase out the use of coal-fired generation.
Many other electricity systems today also have quite a diverse generation mix, with natural gas, coal, nuclear, hydro, biomass, wind and solar PV. A further increase in VRE combined with a decline in conventional generation will require a review of electricity security frameworks by policy makers, supported by input from the wider industry. VRE and other flexibility sources such as demand response and energy efficiency provide an important contribution to adequacy. Fully incorporating these resources into a reliability framework and optimising them in system operations calls for strengthened analysis and appropriate regulatory and market reforms. Early steps in the clean energy transition of particular regions provide critical lessons for those still in an initial phase of their own transition. For the advanced regions, implementation of the next steps is likely to be more challenging than those already achieved.
The significant concentration of low-carbon generation in a few VRE sources, such as onshore wind and PV, will create increasing challenges for policy makers, regulators and system operators. For example:
- The close correlation of VRE generation in certain periods erodes the economic value of energy at these times, potentially creating investment challenges for continued deployment. Additionally, the marginal carbon emission savings will tend to reduce, as higher-emitting energy sources are displaced and competition between low-carbon technologies becomes more common.
- Systems will need to be able to handle increased variability and uncertainty, increasing the need for flexibility.
Tapping into a larger set of variable resources with different generation patterns will reduce these underlying challenges by smoothing the combined VRE output over time, which can both decrease the economic cost of decarbonising the system and soften the integration challenges associated with few generation sources. For instance, solar and wind generation often exhibit both diurnal and seasonal complementarity, reducing the overall variability of VRE output across the day as well as through the year.
For Europe, the growing prospects of offshore wind are a promising opportunity to further diversify the low-carbon mix, as larger capacity factors and complementary generation patterns will soften the integration challenges of VRE. Still, in highly decarbonised systems with diminished nuclear and fossil fleets, other low-carbon sources such as biomass, biogas, hydrogen and carbon capture, use and storage will eventually be needed to cover periods of low VRE generation, together with new flexibility sources such as power storage and the increasing scope of demand-side response.
Maintaining reliability
Lower than needed levels of investment in power systems today present risks for tomorrow
Although wind and solar PV have seen impressive growth in recent years, overall spending in the power sector appears to be less than what will be needed to meet forthcoming security challenges. To this end, the IEA World Energy Investment Report 2020 portrays a rather grim picture. Global investment in the energy sector declines by 20% or USD 400 billion in 2020 in the aftermath of the Covid-19 crisis. The oil and gas upstream sectors see the largest negative impact compared to the electricity sector. Nevertheless, the overall level of investment in power in 2020 declines by 10%. The crisis is prompting a further 9% decline in estimated global spending on electricity networks, which had already fallen by 7% in 2019. Alongside a slump in approvals for new large-scale dispatchable low-carbon power plants (the lowest level for hydropower and nuclear this decade), stagnant spending on natural gas plants and a levelling off in battery storage investment in 2019, these trends are clearly misaligned with the future needs of sustainable and resilient power systems.
Average annual global power sector investment, 2010-2020, and needs to 2040
OpenLow investment levels are projected not only with respect to the requirements of the Sustainable Development Scenario, but also in Stated Policy Scenario pathways. While this has not led immediately to serious power supply incidents, longer-term risks need to be addressed now. Further acceleration can be expected in the deployment of VRE sources like wind and solar PV, due to continuing cost declines and government support schemes. Incentives for flexibility from other parts of the electricity system, including grids, demand response and batteries, receive less focus or only indirect attention in policies and regulatory frameworks worldwide, but are, nevertheless, essential.
The case of European and US electricity markets is very illustrative in this sense. In the past decade following the financial crisis, advanced market economies have seen weaker-than-expected growth in electricity demand in general. Due to a combination of a weak economic recovery, stronger policies on energy efficiency and a rapid spread of efficient technologies like LED lights, electricity demand has stagnated or even declined across all advanced economy systems. By 2015 electricity demand in Europe and the United States was 435 TWh (6.2%) lower than initial expectations for recovery after the financial crisis. This had important and positive implications for electricity security: there was a major wave of investment into combined-cycle gas turbine capacity just before the acceleration of wind and solar PV capacity additions. These gas turbine plants were envisaged as running at a reasonably high load factor to supply robust demand growth. Despite this not materialising, they still have the technical capacity for low and flexible utilisation, primarily providing grid services, which they have done as the share of variable renewables increased. Many jurisdictions have implemented changes to their market design, such as Capacity Remuneration Mechanisms or scarcity pricing, as means to recognise the contribution of these resources to security of supply and attract investments to them.
This example is relevant to the electricity security discussion. System operators and markets succeeded in maintaining robust electricity security while the share of variable renewables grew faster than expected. However, this task was greatly facilitated by the large excess capacity of predominantly flexible units. It should be emphasised, however, that this capacity balance was not the result of a conscious design; rather, it was the result of an unexpected structural break. It also led to massive-scale value destruction as utilities wrote down assets and their equity capitalisation depreciated. The combination of weak demand and value destruction of flexible assets shaped investor expectations, creating a reluctance to invest in these assets.
Future policy and technology changes can also trigger structural breaks. However, there is no guarantee that these will similarly lead to lower-than-expected demand. On the contrary, the coming decades of the electricity transition might well lead to a re‑acceleration of electricity demand growth and a substantially higher generation capacity need, in particular due to a trend of increased electrification.
Technological progress has been highly asymmetrical: low-carbon generating technologies like wind and solar PV, and the technologies enabling electrification such as electric car batteries, have progressed more swiftly and witnessed larger-scale deployment than non-electrical low-carbon options like biofuels. In the previous decade, energy efficiency progress compounded the effect of weaker-than-expected economic growth, leading to surprisingly low power demand.
In the next decade, while the macroeconomic downside risk is unfortunately real, electrification might well outweigh efficiency gains; a household buying an electric car on average adds as much electricity demand as dozens of families replacing refrigerators with ultra-efficient models. The impact of direct electrification would be reinforced by an increasing strategic interest in electrolytic hydrogen, which could replace fossil-fuelled end uses such as heavy trucks or industrial heat. The recently announced EU hydrogen strategy targeting 10 million tonnes of green hydrogen by 2030 would require over 10% of the region’s present electricity generation, which equals the total growth in electrical output during 2000-2010 in a context of stagnation in electricity demand in the recent decade.
In addition to electrification and reaccelerating demand growth, renewables deployment will also have to cover accelerating and nearly unavoidable coal and nuclear capacity decommissioning in many advanced economies. After the value destruction of the past decade, there is little investment appetite for new conventional flexible assets in most mature energy systems. In any case, these may not always be aligned with a credible low-carbon strategy, as is the case for coal. New flexibility enablers from batteries, wider demand response, deeper interconnection of regional systems, new business models and market designs need to fill the gap.
The reliability services required to keep the lights on will need to evolve in response
In addition to controlling total system costs and ensuring necessary investment happens at sufficient pace, policy makers need to take into account that a future low-carbon mix requires dedicated action to ensure a secure system. Regardless of whether countries follow the STEPS or SDS, the share of VRE will be high at a global level and very high in many regions. This implies technical and economic challenges fundamentally different from the ones power systems have faced traditionally.
The integration of VRE can be classified into six phases that capture the evolving impacts, relevant challenges and priority of system integration tasks to support the growth of VRE. While a system will not transition sharply from one phase to the next, the phased categorisation framework can help to prioritise institutional, market and technical measures. For example, issues related to flexibility will emerge gradually in Phase 2 before becoming the hallmark of Phase 3.
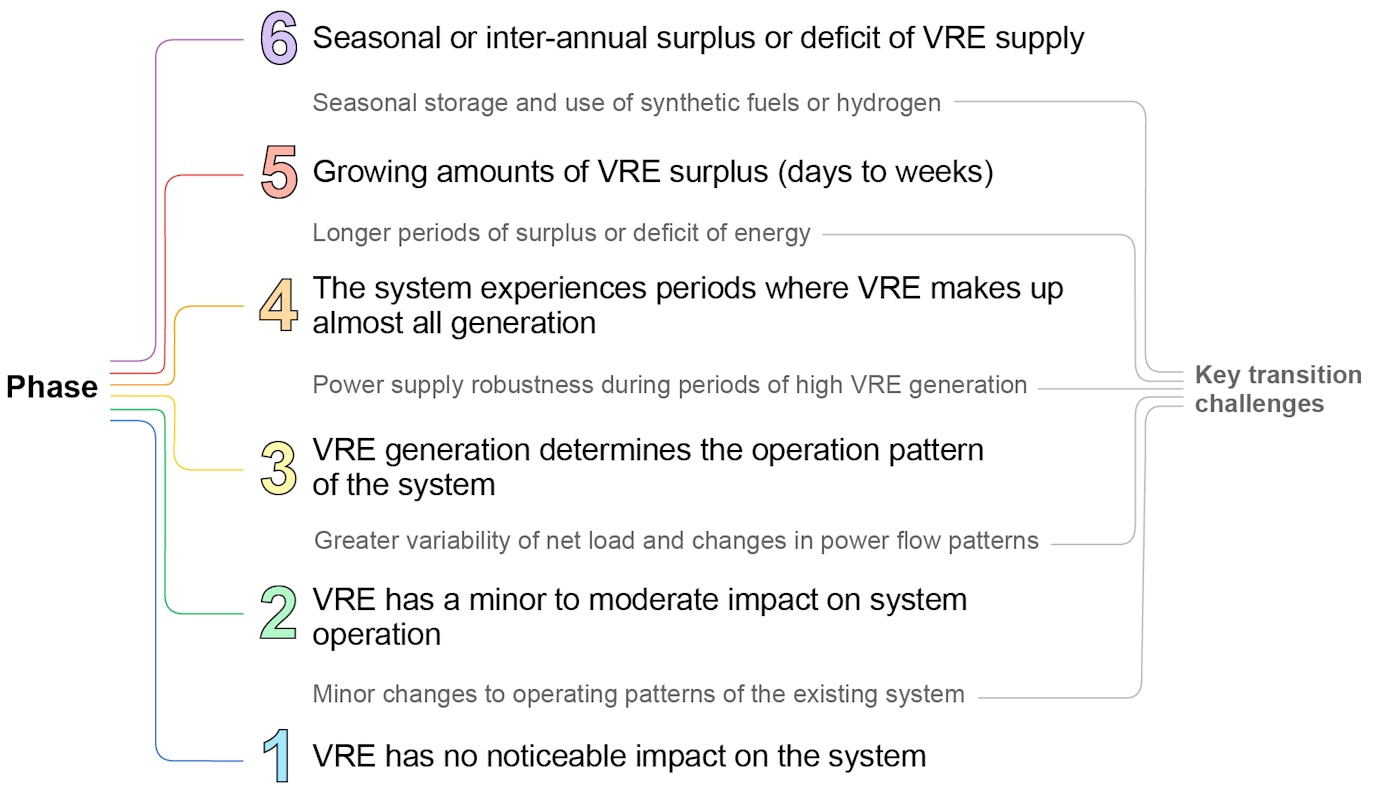
Source: IEA Status of Power System Transformation 2019.
Most systems are still in Phases 1 to 3 and have up to 10-20% share of VRE in annual electricity production. The general trend is clear that higher phases of system integration are forthcoming for most countries. Many countries are expected to enter Phase 4 in the coming years.
Some steps are operational in nature, particularly from Phase 4 as the system faces multiple periods with high levels of VRE generation. While any system has its unique characteristics and legacy, exchange of best practices can support progress in many regions. Inertia provides an illustration of this.
A move to solar PV and wind implies a shift from conventional rotating generation to inverter-based generation. Thermal and hydro generation are based on synchronous machines with heavy rotational mass, which provides inertia to the system. In Phase 4, with a high share of VRE, the system faces challenges in maintaining stability. Inertia is a key parameter in system stability. Solar PV and wind generation, but also electric vehicle chargers, batteries and high-voltage direct-current connections, are all inverter based and do not inherently provide inertia. Technology does allow for a variety of very rapid responses to the needs of the system, known as synthetic inertia. Smaller systems (industrial sites and islands) are already capable of operating at very high levels of VRE infeed. But if large-scale systems such as the extent of continental Europe were to be operated with very high instantaneous solar PV or wind penetration (levels above 70-80%, reached in Phase 4), either new technical inverter capabilities would need to prove their effectiveness or additional investment in synchronous condensers would be needed.
Ensuring the availability of sufficient dispatchable resources has become the major test for ensuring power system reliability
While coal and oil are increasingly pushed out of the system because of carbon emissions and related financing constraints, the role of the other main fossil fuel generation technology – gas – remains strongly debated. New gas plants often take a prominent place in electricity mix scenarios as a flexible resource that can be commissioned in relatively short time frames and that has a lower carbon footprint compared to coal and oil alternatives. Power-to-gas technologies also have the potential to further reduce or eliminate net CO2 emissions from gas generation by using green gas as a fuel. This is without prejudice to the role of biomass (upscaling limitations), nuclear energy (not cost-effective at lower utilisation) or hydro (geographical constraints and resource limitations) in general. Public debate often centres around diverging opinions on whether gas is needed in a low-carbon mix, and whether it is a transition fuel or an impediment to ever reaching a fully decarbonised electricity system.
Policy makers should keep several considerations in mind. Bringing the net emissions of the entire economy to acceptable levels in time may not necessarily preclude a very limited share of emissions in the electricity system. Flexible gas peaker plants can provide security to a low-carbon system in a viable manner if the very low number of full load hours are remunerated accordingly. But low-carbon scenarios that rely on gas peaker plants should not expect such plants to materialise spontaneously under present market conditions. Acknowledging the role of gas (or other) fossil fuel plants in a secure low-carbon mix should be focused on ensuring adequacy and complement and facilitate the deployment of low-carbon technologies. Policy debate rightfully needs to scrutinise whether capacity remuneration mechanisms that are open to carbon-intensive sources are designed and implemented in such way.
Evidently allowing for some level of carbon emissions in the electricity system increases the burden on other already hard-to-abate sectors such as industry and transport. This makes it a fundamental policy question as it balances efforts in other sectors and considers an element of risk hedging. Future breakthroughs in battery storage, the viability of renewable synthetic fuels and the scaling up of demand flexibility may eventually reduce or replace the need for gas plants as known today, or they may not.
Technology shifts
Reliance on gas for generation adequacy creates an intimate link between electricity supply security and gas deliverability
In many electricity systems the energy transition goes hand in hand with a rising share of variable renewables (wind and solar) and reduced fuel diversity of flexible electricity generation (due to coal, lignite and nuclear phase-outs). This increases the reliance of the power system on gas-fired power plants during peak demand with simultaneously low wind and solar generation.
Consequently, the role of gas-fired power plants for providing supply flexibility will become increasingly important, creating a more intimate link between security of electricity supply and natural gas deliverability. This makes it necessary to assess the deliverability of the gas system, especially when considering declining domestic production and potential closures of gas storage sites.
The European Union’s energy system will see an acceleration of the transition described above over the next decade, with solar PV and wind doubling their current annual supply, whilst coal and nuclear generation will practically halve during the same period.
Gas-fired generation will become crucial to meet peak demand in extreme weather events – about 85% higher than during the peak in 2018, with a corresponding increase in demand for natural gas.
North west Europe’s peak supply days in 2018 compared with 2030
OpenDigitalisation will come to the fore
Policy makers will need to update their catalogue of technologies that contribute to security of supply and properly address their contribution. They will also need to take advantage of the increased capabilities brought by digitalisation to keep the cost of security of supply down while we electrify other final uses of energy, such as transport and heating.
From an operational point of view, digitalisation and new capabilities brought by technologies such as batteries will expand the toolbox that policy makers and system operators can use to maintain security of supply. Increasing digitalisation has the power to mobilise an array of end-use devices to respond to the power sector’s needs, increasing or reducing loads at times of distress. Some power systems, such as in Great Britain, already use batteries storage devices to minimise the damage of sudden outages, injecting almost instantaneously the power needed to avoid cascade events. This was one of the resources that prevented a full‑black system event in August 2019. Distributed resources can also be used to support recovery in a black system event and provide a basic level of supply to essential services in cases where towns are electrically “islanded”. Technology is already capable of providing many services, and regulation, interconnection standards and grid codes will require updating to take advantage of all the capabilities of existing assets.
In the coming years, deep electrification will require us to take advantage of digitalisation to control an array of electrical devices could reduce their consumption following price signals or technical orders linked to stress on the system.
With the exception of load shedding and calls to voluntarily reduce their consumption, customers have been isolated from almost any interaction related to relieving stress on the system. Following the same approach with high levels of transport and heating electrification would be onerous and wasteful, as technology is already capable of controlling an array of non-critical devices via external telecommunications protocols.
New technologies like storage, VRE and electric vehicles will change our concept of security of supply
This new stocktaking will also be essential to ensure the long-term availability of resources and adequacy. Larger interconnected electricity systems can smooth out part of the variability of demand and solar PV and wind generation by using geographical diversification. New methodologies are needed to assess the adequacy of a large-scale system with variable sources and more active demand fluctuations. Policy makers need to take into account the extent of the smoothing effect in an interconnected system.
Wind and solar PV outputs have some complementary patterns in particular regions. Offshore wind specifically has relatively high capacity factors, suggesting the extent to which it can be dispatched to follow demand patterns. Large areas see more stable wind generation compared to individual sites. There is, however, a limit to such smoothing effects. Dispatchable generation, storage and more intelligent use of grids and flexible demand will become essential at some point. Extreme and rare situations also need to be planned for, as illustrated in Japan where a large-scale typhoon would cause a major proportion of wind turbines in the country to become unavailable at the same time.
Battery technologies and demand response are growing in capacity and capability, and can play a substantial role in integrating variable renewable generators. These options are effective in providing short-term flexibility and absorbing fluctuations on a minute or hourly basis, although they are not suitable for medium- to long-term energy storage (weeks to months). Many countries experience several consecutive days of low wind speed or rain and heavy cloud. Such weather conditions have not become major obstacles for power supplies thanks to contributions from other flexible power generation sources and electricity trade with neighbouring regions. Reservoir and pumped storage hydropower are an important source of short- and medium-term energy storage in regions with resource availability. Adding new pumped storage capacity to existing facilities can be an avenue for development where existing resource potential is largely exploited.
Emerging technologies, such as power to hydrogen or biomethanation, have the potential to serve as sources of long-term flexibility, including seasonal. There is, however, still the need for technological development to de-risk their application on a commercial scale if they are to provide the seasonal storage buffer that high-VRE systems need when they enter Phases 5 and 6. These challenges are subject to today’s technological availability. Breakthroughs in various key technologies could foster secure electricity supply with very high shares of wind and solar PV in the coming years at a reasonable cost. As long as innovation uncertainty exists in the power system transformation, however, realistic solutions need to be pursued as a technological hedge against such uncertainty. Expanding variable renewable deployment and ramping up innovation efforts are no-regret actions that are essential to steer our energy trajectory onto a sustainable and secure future path. In parallel, governments should make maximum effort to securing diversity in their low-carbon power generation mix by considering measures such as:
- developing hydropower sources, including the refurbishment and upgrade of existing plants to include pumped storage options where possible
- maintaining existing nuclear capacity by lifetime extensions and continuing research into new technologies such as small modular reactors
- expanding the use of sustainable biofuels and biogas in power generation
- pursuing a true transition to synthetic and low-carbon fuels and gases.
A technology-neutral approach should be adopted in policy measures to establish a low-carbon electricity system. And power market designs need to reward not only energy, but also flexibility and adequacy contributions to the entire system. During the period when technological progress or capital resources do not allow for the full decarbonisation of the electricity system, using coal- or gas-fired power plants solely for flexibility purposes or emergency reserves could be options to take advantage of their capacity, if this fits into a wider energy and economy decarbonisation strategy.
From a purely technical and cost perspective, all fuels and all technologies should be considered for achieving a sustainable energy path in all sectors. But the electricity generation mix should lead the way since it will still be the major source of flexibility.
New planning tools and incentives
New technologies need new planning tools. Probabilistic analysis provides better security-of-supply assessment
Policy makers, regulators and system operators can improve the accuracy of their adequacy assessments by moving away from deterministic methods, such as planning reserve margin, and developing probabilistic simulations of the variability and interdependence of outcomes in their systems. Through simulations, decision makers gain a deeper understanding of system elements by valuing their contribution to adequacy, including:
- VRE variability
- outages for generators and transmission lines
- regional interconnection availability
- system reserve margins and main system contingencies
- load variability
- demand response.
In a simulation approach, generation and transmission outage statistics can be combined with a detailed representation of the physical topology of the system. Possible outcomes can be simulated for many different random patterns of outages of all technologies. This provides a more accurate answer compared to considering each piece of infrastructure separately, and it gives a picture of the statistical spread of possible outcomes.
Simulations also allow for deepening the analysis further, for example to account for time-based variation of the likelihood of generator or transmission outage based on the local weather conditions. Larger regions can also be represented, allowing the reliability contribution of interconnected areas to be understood. Different areas may be stressed under similar conditions, in which case a limited contribution to reliability is a given. However, as areas typically have a different energy mix and load profile, and see a smoothing effect in aggregated VRE, interconnections will often provide increased adequacy. Operating reserve margins are another area that deserves close consideration, including dynamic reserve sizing that takes into account load, variable generation and the largest contingencies in the system.
Market design should translate stress periods in the power system into higher wholesale prices
In an evolving system, the services needed to retain security of supply will also evolve. It is more important than ever to verify that the participants in the power sector are given the right incentives to supply not only clean energy, but also services such as net peak load capacity, flexibility and reserves.
To ensure secure operation, governments and regulators should define an acceptable level of supply interruption. While doing this, policy makers must consider the value that customers place on electricity, and the economic and social impact of limited outages. This is the role of reliability standards. Then regulators need to create market and investment frameworks that can bring the desired level of security. Markets have to deliver not only peak capacity as in traditional power systems, but also all the services needed to ensure secure operation of the system.
Energy-only, energy plus capacity and centrally planned systems approach the incentives for achieving the desired level of security by either setting them administratively or leaving them to market forces. A major point of attention for policy makers in any of the three approaches is to ensure that all forms of flexibility in the system are recognised and can provide system services in an economically sensible manner to minimise overall system costs.
This is particularly relevant when dispatchable capacity expects to be used infrequently – the asset’s fixed costs must be compensated either through the margin gained on energy sales during the relatively small number hours in which the unit operates or provides reserves, or through a capacity payment. The energy-only option needs to allow strong energy price signals to avoid the “missing money” problem that occurs when price caps discourage worthwhile investments. Alternatively, whenever a decision is taken to add capacity mechanisms in a liberalised market, careful consideration needs to be given to all possible cost-related impacts, the true verifiable reliability improvements and possible lock-in situations.